Radha
Tuesday, December 17, 2013
Thursday, August 29, 2013
Saturday, July 13, 2013
Tuesday, July 09, 2013
Monday, April 29, 2013
Stars: Formation, Classification and Constellations
Stars are giant, luminous spheres of plasma. There are billions of them — including our ownsun — in the Milky Way Galaxy. And there are billions of galaxies in the universe. So far, we have learned that hundreds also have planets orbiting them.
History of Observations
Since the dawn of recorded civilization, stars played a key role in religion and proved vital to navigation. Astronomy, the study of the heavens, may be the most ancient of the sciences. The invention of the telescope and the discovery of the laws of motion and gravity in the 17th century prompted the realization that stars were just like the sun, all obeying the same laws of physics. In the 19th century, photography and spectroscopy — the study of the wavelengths of light that objects emit — made it possible to investigate the compositions and motions of stars from afar, leading to the development of astrophysics. In 1937, the first radio telescope was built, enabling astronomers to detect otherwise invisible radiation from stars. In 1990, the first space-based optical telescope, the Hubble Space Telescope, was launched, providing the deepest, most detailed visible-light view of the universe.
Star Naming Designations
Ancient cultures saw patterns in the heavens that resembled people, animals or common objects — constellations that came to represent figures from myth, such as Orion the Hunter, a hero in Greek mythology. Astronomers now often use constellations in the naming of stars, with the International Astronomical Union, the world authority for assigning names to celestial objects, officially recognizing 88 constellations that cover the entire sky. Usually, the brightest star in a constellation is has "alpha," the first letter of the Greek alphabet, as part of its scientific name. The second brightest star in a constellation is typically designated "beta," the third brightest "gamma," and so on until all the Greek letters are used, after which numerical designations follow. See our overview of Constellations.
Since there are so many stars in the universe, the IAU uses a different system for newfound stars. Most consist of an abbreviation that stands for either the type of star or a catalog that lists information about the star, followed by a group of symbols. For instance, PSR J1302-6350 is a pulsar, thus the PSR. The J reveals that a coordinate system known as J2000 is being used, while the 1302 and 6350 are coordinates similar to the latitude and longitude codes used on Earth.
A number of stars have possessed names since antiquity — Aldebaran, for instance, means "the follower" in Arabic, as it seems to follow the Pleiades, or Seven Sisters star cluster, across the sky. These possess scientific names as well — Aldebaran is also known as Alpha Tauri.A young, glittering collection of stars looks like an aerial burst. The cluster is surrounded by clouds of interstellar gas and dust—the raw material for new star formation. The nebula, located 20,000 light-years away in the constellation Carina, contains a central cluster of huge, hot stars, called NGC 3603.
Formation
A star develops from a giant, slowly rotating cloud that is made up entirely or almost entirely of hydrogen and helium. Due to its own gravitational pull, the cloud behind to collapse inward, and as it shrinks, it spins more and more quickly, with the outer parts becoming a disk while the innermost parts become a roughly spherical clump. This collapsing material grows hotter and denser, forming a ball-shaped protostar. When the heat and pressure in the protostar reaches about 1.8 degrees F (1 million degrees C), atomic nuclei that normally repel each other start fusing together, and the star ignites. Nuclear fusion converts a small amount of the mass of these atoms into extraordinary amounts of energy — for instance, 1 gram of mass converted entirely to energy would be equal to an explosion of roughly 22,000 tons of TNT.
Evolution. The life cycles of stars follow patterns based mostly on their initial mass. These include intermediate-mass stars such as the sun, with half to eight times the mass of the sun, high-mass stars that are more than eight solar masses, and low-mass stars a tenth to half a solar mass in size. The greater a star's mass, the shorter its lifespan generally is. Objects smaller than a tenth of a solar mass do not have enough gravitational pull to ignite nuclear fusion — some might become failed stars known as brown dwarfs.
An intermediate-mass star begins with a cloud that takes about 100,000 years to collapse into a protostar with a surface temperature of about 6,750 degrees F (3,725 degrees C). After hydrogen fusion starts, the result is a T-Tauri star, a variable star that fluctuates in brightness. This star continues to collapse for roughly 10 million years until its expansion due to energy generated by nuclear fusion is balanced by its contraction from gravity, after which point it becomes a main-sequence star that gets all its energy from hydrogen fusion in its core.
The greater the mass of such a star, the more quickly it will use its hydrogen fuel and the shorter it stays on the main sequence. After all the hydrogen in the core is fused into helium, the star changes rapidly — without nuclear radiation to resist it, gravity immediately crushes matter down into the star's core, quickly heating the star. This causes the star's outer layers to expand enormously and to cool and glow red as they do so, rendering the star a red giant. Helium starts fusing together in the core, and once the helium is gone, the core contracts and becomes hotter, once more expanding the star but making it bluer and brighter than before, blowing away its outermost layers. After the expanding shells of gas fade, the remaining core is left, a white dwarf that consists mostly of carbon and oxygen with an initial temperature of roughly 180,000 degrees F (100,000 degrees C). Since white dwarves have no fuel left for fusion, they grow cooler and cooler over billions of years to become black dwarves too faint to detect. (Our sun should leave the main sequence in about 5 billion years.)
A high-mass star forms and dies quickly. These stars form from protostars in just 10,000 to 100,000 years. While on the main sequence, they are hot and blue, some 1,000 to 1 million times as luminous as the sun and are roughly 10 times wider. When they leave the main sequence, they become a bright red supergiant, and eventually become hot enough to fuse carbon into heavier elements. After some 10,000 years of such fusion, the result is an iron core roughly 3,800 miles wide (6,000 km), and since any more fusion would consume energy instead of liberating it, the star is doomed, as its nuclear radiation can no longer resist the force of gravity. When the iron core of such a star reaches a mass of 1.4 solar masses, the result is a supernova. Gravity causes the core to collapse, making the core temperature rise to nearly 18 billion degrees F (10 billion degrees C), breaking the iron down into neutrons and neutrinos. In about one second, the core shrinks to about six miles (10 km) wide and rebounds just like a rubber\ ball that has been squeezed, sending a shock wave through the star that causes fusion to occur in the outlying layers. The star then explodes in a so-called Type II supernova. If the remaining stellar core was less than roughly three solar masses large, it becomes a neutron star made up nearly entirely of neutrons, and rotating neutron stars that beam out detectable radio pulses are known as pulsars. If the stellar core was larger than about three solar masses, no known force can support it against its own gravitational pull, and it collapses to form a black hole.
A low-mass star uses hydrogen fuel so sluggishly that they can shine as main-sequence stars for 100 billion to 1 trillion years — since the universe is only about 13.7 billion years old, this means no low-mass star has ever died. Still, astronomers calculate these stars, known as red dwarfs, will never fuse anything but hydrogen, which means they will never become red giants. Instead, they should eventually just cool to become white dwarfs and then black dwarves.
Binary stars and other multiples.
Although our solar system only has one star, most stars like our sun are not solitary, but are binaries where two stars orbit each other a pair, or multiples involving even more stars. In fact, just one-third of stars like our sun are single, while two-thirds are multiples — for instance, the closest neighbor to our solar system, Proxima Centauri, is part of a multiple system that also includes Alpha Centauri A and Alpha Centauri B. Still, class G stars like our sun only make up some 7 percent of all stars we see — when it comes to systems in general, about 30 percent in our galaxy are multiple, while the rest are single.
Binary stars develop when two protostars form near each other. One member of this pair can influence its companion if they are close enough together, stripping away matter in a process called mass transfer. If one of the members is a giant star that leaves behind a neutron star or a black hole, an X-ray binary can form, where matter pulled from the stellar remnant's companion can get extremely hot and emit X-rays. If a binary includes a white dwarf, gas pulled from a companion onto the white dwarf's surface can fuse violently in a flash called a nova. At times, enough gas builds up for the dwarf to collapse, leading its carbon to fuse nearly instantly and the dwarf to explode in a Type I supernova, which can outshine a galaxy for a few months.
Characteristics
Brightness
Astronomers describe star brightness in terms of magnitude and luminosity.
The magnitude of a star is based on a scale more than 2,000 years old, devised by Greek astronomer Hipparchus in about 125 BC. He numbered groups of stars based on their brightness as seen from Earth — the brightest ones were called first magnitude stars, the next brightest were second magnitude, and so on up to sixth magnitude, the faintest visible ones. Nowadays astronomers refer to a star's brightness as viewed from Earth as its apparent magnitude, but since the distance between Earth and the star can affect the light one sees from it, they now also describe the actual brightness of a star using the term absolute magnitude, which is defined by what its apparent magnitude would be if it were 10 parsecs or 32.6 light years from Earth. The magnitude scale now runs to more than six and less than one, even descending into negative numbers — the brightest star in the night sky is Sirius, with an apparent magnitude of -1.46.
Luminosity is the power of a star — the rate at which it emits energy. Although power is generally measured in watts — for instance, the sun's luminosity is 400 trillion trillion watts— the luminosity of a star is usually measured in terms of the luminosity of the sun. For example, Alpha Centauri A is about 1.3 times as luminous as the sun. To figure out luminosity from absolute magnitude, one must calculate that a difference of five on the absolute magnitude scale is equivalent to a factor of 100 on the luminosity scale — for instance, a star with an absolute magnitude of 1 is 100 times as luminous as a star with an absolute magnitude of 6.
The brightness of a star depends on its surface temperature and size.
Color
Stars come in a range of colors, from reddish to yellowish to blue. The color of a star depends on surface temperature.
A star might appear to have a single color, but actually emits a broad spectrum of colors, potentially including everything from radio waves and infrared rays to ultraviolet beams and gamma rays. Different elements or compounds absorb and emit different colors or wavelengths of light, and by studying a star's spectrum, one can divine what its composition might be.
Surface temperature
Astronomers measure star temperatures in a unit known as the kelvin, with a temperature of zero K equaling minus 273.15 degrees C, or minus 459.67 degrees F. A dark red star has a surface temperature of about 2,500 K (2,225 degrees C and 4,040 degrees F); a bright red star, about 3,500 K (3,225 degrees C and 5,840 degrees F); the sun and other yellow stars, about 5,500 K (5,225 degrees C and 9,440 degrees F); a blue star, about 10,000 K (9,725 degrees C and 17,540 degrees F) to 50,000 K (49,725 degrees C and 89,540 degrees F).
The surface temperature of a star depends in part on its mass and affects its brightness and color. Specifically, the luminosity of a star is proportional to temperature to the fourth power. For instance, if two stars are the same size but one is twice as hot as the other in kelvin, the former would be 16 times as luminous as the latter.
Size
Astronomers generally measure the size of stars in terms of the radius of our sun. For instance, Alpha Centauri A has a radius of 1.05 solar radii (the plural of radius). Stars range in size from neutron stars, which can be only 12 miles (20 kilometers) wide, to supergiants roughly 1,000 times the diameter of the sun.
The size of a star affects its brightness. Specifically, luminosity is proportional to radius squared. For instance, if two stars had the same temperature, if one star was twice as wide as the other one, the former would be four times as bright as the latter.
Mass
Astronomers represent the mass of a star in terms of the solar mass, the mass of our sun. For instance,Alpha Centauri A is 1.08 solar masses.
Stars with similar masses might not be similar in size because they have different densities. For instance, Sirius B is roughly the same mass as the sun, but is 90,000 times as dense, and so is only a fiftieth its diameter.
The mass of a star affects surface temperature.
Magnetic field
Stars are spinning balls of roiling, electrically charged gas, and thus typically generate magnetic fields. When it comes to the sun, researchers have discovered its magnetic field can become highly concentrated in small areas, creating features ranging from sunspots to spectacular eruptions known as flares and coronal mass ejections. Directly detecting the magnetic fields of other stars, however, can be difficult.
Metallicity
The metallicity of a star measures the amount of "metals" it has — that is, any element heavier than helium.
Three generations of stars may exist based on metallicity. Astronomers have not yet discovered any of what should be the oldest generation, Population III stars born in a universe without "metals." When these stars died, they released heavy elements into the cosmos, which Population II stars incorporated relatively small amounts of. When a number of these died, they released more heavy elements, and the youngest Population I stars like our sun contain the largest amounts of heavy elements.
Classification
Stars are typically classified by their spectrum in what is known as the Morgan-Keenan or MK system. There are eight spectral classes, each analogous to a range of surface temperatures — from the hottest to the coldest, these are O, B, A, F, G, K, M, and L. Each spectral class also consists of 10 spectral types, ranging from the numeral 0 for the hottest to the numeral 9 for the coldest.
Stars are also classified by their luminosity under the Morgan-Keenan system. The largest and brightest classes of stars have the lowest numbers, given in Roman numerals — Ia is a bright supergiant; Ib, a supergiant; II, a bright giant; III, a giant; IV, a subgiant; and V, a main sequence or dwarf.
A complete MK designation includes both spectral type and luminosity class — for instance, the sun is a G2V.
Stellar Structure
The structure of a star can often be thought of as a series of thin nested shells, somewhat like an onion.
A star during most of its life is a main-sequence star, which consists of a core, radiative and convective zones, a photosphere, a chromosphere and a corona. The core is where all the nuclear fusion takes places to power a star. In the radiative zone, energy from these reactions is transported outward by radiation, like heat from a light bulb, while in the convective zone, energy is transported by the roiling hot gases, like hot air from a hairdryer. Massive stars that are more than several times the mass of the sun are convective in their cores and radiative in their outer layers, while stars comparable to the sun or less in mass are radiative in their cores and convective in their outer layers. Intermediate-mass stars of spectral type A may be radiative throughout.
After those zones comes the part of the star that radiates visible light, the photosphere, which is often referred to as the surface of the star. After that is the chromosphere, a layer that looks reddish because of all the hydrogen found there. Finally, the outermost part of a star's atmosphere is the corona, which if super-hot might be linked with convection in the outer layers.
Wednesday, March 13, 2013
Tuesday, June 26, 2012
Implications of the laws of Thermodynamics
If there are any scientific laws that have universal acceptance, and universal applicability, they are the laws of thermodynamics. Thermodynamics literally means "energy in action." It is a word with roots that indicate that it has to do with both heat and motion. (See here for the Wikipedia article on the first law , and on the second law . There are actually more than two laws, but I won't consider any but the first and second in this document.)
Statements/definitions
The first law of thermodynamics may be stated thus: "energy can neither be created nor destroyed." This law does not rule out transformations of energy from one form to another, or even transformations of energy to matter, and the reverse, as according to Einstein's E=mc2 equation. If it is true, it means that neither energy, nor matter, can be created from nothing. The second law of thermodynamics is more difficult to state in plain English. Here is an attempt: "In any transformation of energy from one form to another, 'useful' energy is lost."The first law is sometimes stated as "you can't get something for nothing." The second law tells us that not only can't we get something for nothing, but that we always lose in the process. We give up more than we get, energetically speaking.
A familiar consequence of that is the fact that much of the energy of automobile engines does not end up in motion, but in overcoming friction. Another is that it is not possible to have a refrigerator, freezer, or air conditioner that merely removes energy from one place to another. In the process of moving energy, each of these actually increases the total heat in the universe, as it uses electricity (or gas, etc.) to do its work, and some of that work is not merely to transfer heat, but to overcome friction. Another consequence is that when light energy is transformed into chemical energy in green plants by photosynthesis, and then to chemical energy in animals that eat green plants, most of the light energy is not actually transformed into chemical energy in animals, but does various "non-useful" things. As a result, meat of all kinds is more expensive than plant food, either in the grocery store, or if you grow your own. To put it another way, you can feed a family on a lot less space, using a garden, than the space required to feed the same family, if they eat only meat.

An ordered system. Entropy is low. There is a concentration of some substance in one area. |
![]() A disordered system. Entropy is high. There isn't a concentration of a substance. |
Consequences
There are important philosophic and religious implications of the laws of thermodynamics. If the first law is true, and has always been true, then the universe has always existed. If the second law is true, and has always been true, then if the universe has always existed, by this "time" it would have achieved complete entropy. If you don't understand these statements, read the first section over again, carefully. Obviously these statements are not both true. If they were, the universe would be uninhabitable. How to explain this? The only explanation is that laws of thermodynamics haven't always been true. Why not? One possibility is that there was intervention by a supernatural God, creating something from nothing, in the initial creation of the universe. Another way to say this is that the universe had a definite beginning. There was a time when it did not exist, at least in its present form, then God brought it into being. It is impossible to prove or disprove this, scientifically. However, it is a solution to the dilemma posed in the italicized statements at the beginning of this paragraph.
Another solution to the dilemma is to say that the "big bang" (That is, a giant explosion that changed the universe from some original state to the present one, at some specific time in the distant past) started the universe, or started it over. The two solutions, that God began the universe, or that there was a big bang, are not necessarily contradictory. Many Christian scientists believe that God used the big bang to start the universe as we know it. The big bang is not an explanation, by itself. It merely puts the question one step back. What caused the big bang? (Referring to God also leads to a question one step back, namely "Where did God come from?" The usual explanation is that God has always existed; He is eternal.) It seems to me that any explanation of the beginning of the universe depends on ideas that cannot be proved, that require faith , in other words.
In his The Unconscious Quantum (Amherst, New York: Prometheus Books, 1995) Victor J. Stenger says that "In fact, our best current estimate of the total energy of the universe, including the rest energy of matter, is essentially consistent with zero. Within observational accuracies, the rest and kinetic energies of the material bodies of the universe are almost exactly balanced by the negative potential energy of their gravitational interactions." (p. 219) He goes on to say that this includes so-called dark matter, and to explicitly indicate that, if the total energy in the universe is now zero, no violation of the first law of thermodynamics would have been necessary at the beginning of the universe. Dark matter is beyond the scope of the courses I teach, which is a good thing, because I don't understand it. (I'm not sure anyone does.) However, even if Stenger is correct, (which would make an argument for a creator from the laws of thermodynamics weaker, or nullify it entirely) three points can be raised. 1) Why and how did the universe arise from nothing? 2) What he says does not rule out God's activity, at the beginning, and now. 3) There has still been a very long time for the second law to operate, if the beginning of the universe took place as far back as standard scientific theory says it did. For more on this subject, see the Energy of the Cosmos section of the Wikipedia article on Physical Cosmology. I include this paragraph because I want to acknowledge views which differ with mine. I am not sure how well Stenger represents the part of the scientific community with expertise in this area. A review of this book , by an atheist with physics qualifications, indicates that he is obsessively against anything that hints of the spiritual.
The Resurrection
. . . and his incomparably great power for us who believe. That power is like the working of his mighty strength, which he exerted when he raised him from the dead and seated him at his right hand in the heavenly realms . . . (Ephesians 1:19-20, NIV) |
In our present world, change and decay are built into the fabric of the universe. The processes by which genetic mutations produce new forms of life are the processes by which cells become cancerous. Death is the necessary cost of life. In fact, a theological defense of the existence of physical evil is that it is not gratuitous but the inescapable price of an evolutionary world, free to make itself within the independence its Creator has granted to it. John C. Polkinghorne, Serious Talk: Science and Religion in Dialogue. Harrisburg, PA: Trinity Press International, 1995, p. 107. |
Diet (Note also the fourth paragraph in this document, which deals with plant vs. animal food.) Body processes often transform energy from one form to another. When this happens, according to the second law, useful energy is lost. As a result, even adults must have a source of energy to live on. The only significant source of energy for humans is the food they eat. By the laws of thermodynamics, there are three possibilities. If you take in less useful energy than you lose, you will lose energy (calories). Since calories are stored in material, chemical, form, that means that you will lose weight. If you take in more energy than you lose, you will gain calories and weight. If you take in the same amount, on the average, you will maintain your current calorie amount and weight.
Temperature and Heat
Temperature is a measure of the velocity of the average molecule. In other words, when the temperature is 100 any molecules that can move easily are moving considerably faster than they would be at 50. At absolute zero, all molecular motion would cease. (Actually, absolute zero has never been detected. It is possible to come close to it in laboratory situations, and some remarkable things happen when you do. Absolute zero is about -473o Fahrenheit.)
Nuclear Fusion
Please remember that when a system gives off energy, it becomes more stable. This is true of atomic nuclei, too. The mass of a neutron is 1.008665 atomic mass units. (amu) The mass of a proton is 1.007276 amu. The mass of a Helium4 nucleus is 4.002603 amu. The total mass of the 2 neutrons and 2 protons which make up a Helium4 nucleus is 4.031882 amu. What happened to the remaining 0.029279 amu? It was converted to energy according to Einstein’s E=mc2 equation. This is the binding energy. Since there are 4 nucleons in He4, the binding energy per nucleon is equivalent to 0.00731975 amu = 0.029279amu/4. This energy was given up in the formation of the Helium4 nucleus.
Consider a pile of kindling wood and enough matches to light it, versus the pile of ashes that would be formed if it were burned. Which is most stable? The ashes. Which would be more stable, partially burned or totally burned wood? Totally burned wood. Which would have given off more energy? Totally burned wood. Binding energy and burning can be compared. The more binding energy that is given off, the more stable a nucleus will be.
I indicated above that Helium4 has a binding energy, and that mass is lost in the formation of He4 from protons and neutrons. Helium4 can be made from Hydrogen1. Most of the energy of the sun comes from just that reaction. The temperature of the interior of the sun is estimated to be in the millions. One consequence of that is that high temperature is that electrons almost always leave their nuclei entirely—they are just sort of running madly around, not attached to anything. Another result of the high temperature is that the remaining Hydrogen nuclei, or protons, are moving so rapidly that they can overcome the repulsion between two positive objects, and combine into a single entity. In the process, one of them becomes a neutron. This new nucleus, H2, has given off binding energy. Actually, the process continues with further proton capture, and eventually a He4 nucleus is formed. This is even more stable than H2. Some of the energy given off by this process escapes the sun, and it is a good thing, because that energy is the energy which living things on earth depend on.
The sun is not burning in the usual sense. The energy of the sun comes from nuclear fusion.
Note - my source of data is Modern Physics, by Kenneth Krane. (New York: Wiley, 1983)

This work is licensed under a Creative Commons License . The purpose of this is NOT to prevent use by others, but to prevent other users from restricting free use. I claim no ownership of materials in the above material which are quotations from other sources.

Thursday, November 24, 2011
Friday, July 22, 2011
Sunday, July 10, 2011
Monday, July 04, 2011
Mathematicians Want to Say Goodbye to Pi
"I know it will be called blasphemy by some, but I believe that pi is wrong."
That's the opening line of a watershed essay written in 2001 by mathematician Bob Palais of the University of Utah. In "Pi is Wrong!" Palais argued that, for thousands of years, humans have been focusing their attention and adulation on the wrong mathematical constant.
Two times pi, not pi itself, is the truly sacred number of the circle, Palais contended. We should be celebrating and symbolizing the value that is equal to approximately 6.28 — the ratio of a circle's circumference to its radius — and not to the 3.14'ish ratio of its circumference to its diameter (a largely irrelevant property in geometry).
Last year, Palais' followers gave the new constant, 2pi, a name: tau. Since then, the tau movement has steadily grown, with its members hoping to replace pi as it appears in textbooks and calculators with tau, the true idol of math. Yesterday — 6/28 — they even celebrated Tau Day in math events worldwide.
But is pi really "wrong"? And if it is, why is tau better?
The mathematicians aren't saying that pi has been wrongly calculated. Its value is still approximately 3.14, as it always was. Rather they argue that 3.14 isn't the value that matters most when it comes to circles. Palais originally argued that pi should be changed to equal 6.28 while others prefer giving that number a new name altogether.
Kevin Houston, a mathematician at the University of Leeds in the U.K. who has made a YouTube video to explain all the advantages of tau over pi, said the most compelling argument for tau is that it is a much more natural number to use in the fields of math involving circles, like geometry, trigonometry and even advanced calculus.
"When measuring angles, mathematicians don't use degrees, they use radians," Houston enthusiastically told Life's Little Mysteries, a sister site to LiveScience. "There are 2pi radians in a circle. This means one quarter of a circle corresponds to half of pi. That is, one quarter corresponds to a half. That's crazy. Similarly, three quarters of a circle is three halves of pi. Three quarters corresponds to three halves!" [A Real Pie Chart: America's Favorite Pies]
"Let's now use tau," he continued. "One quarter of a circle is one quarter of tau. One quarter corresponds to one quarter! Isn't that sensible and easy to remember? Similarly, three quarters of a circle is three quarters of tau." Making tau equal to the full angular turn through a circle, he said, is "so easy and would prevent math, physics and engineering students from making silly errors."
A better teaching tool
Aside from preventing errors, as Palais put it in his article, "The opportunity to impress students with a beautiful and natural simplification has turned into an absurd exercise in memorization and dogma."
Indeed, other tau advocates have said they've noticed a significant improvement in the ability of students to learn math, especially geometry and trigonometry where factors of 2pi show up the most, when the students learn with tau rather than pi.
Though 2pi appears much more often in calculations than does pi by itself (in fact, mathematicians often accidentally drop or ad that extra factor of 2 in their calculations), "there is no need for pi to be eradicated," Houston said. "You might say I'm not anti-pi, I'm pro-tau. Hence, anyone could use pi when they had a calculation involving half of tau."
Tau, the 19th letter of the Greek alphabet, was chosen independently as the symbol for 2pi by Michael Hartl, physicist and mathematician and author of "The Tau Manifesto," and Peter Harremoës, a Danish information theorist. In an email, Houston explained their choice: "It looks a bit like pi and is the Greek 't,' so fits well with the idea of turn. (Since tau is used in angles you can talk about one quarter turn and so on.)"
Pi is too ingrained in our culture and our math to succumb to tau overnight, but the movement pushes ever onward. "Change will be incremental," Houston said.
Original post: http://news.yahoo.com/mathematicians-want-goodbye-pi-154001699.html
Monday, June 20, 2011
Tuesday, June 14, 2011
Soma
Nothing left to say
And all I've left to do
Is run away
From you
And she led me on, down
With secrets I can't keep
Close your eyes and sleep
Don't wait up for me
Hush now don't you speak
To me
Wrapped my hurt in you
And took my shelter in that pain
The opiate of blame
Is your broken heart, your heart
Soma, I'm all by myself
As I've always felt
I'll betray my tears
To anyone caught in our ruse of fools
One last kiss for me...yeah
One last kiss good night
Didn't want to lose you once again
Didn't want to be your friend
Fulfilled a promise made of tin
And crawled back to you
I'm all by myself
As I've always felt
I'll betray myself
To anyone, lost, anyone but you
So let the sadness come again
On that you can depend on me, yeah
Until the bitter, bitter end of the world, yeah
When god sleeps in bliss
And I'm all by myself
As I've always felt
And I'll betray myself
To anyone
Friday, April 22, 2011
Saturday, April 09, 2011
Saturday, January 08, 2011
Tuesday, September 14, 2010
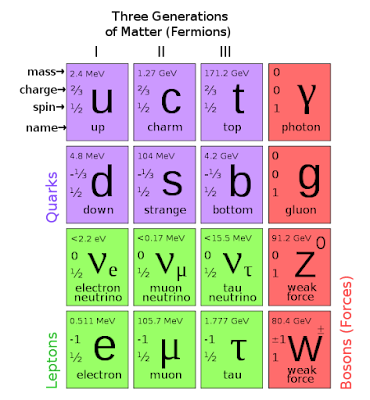
Under the "quarks and leptons" definition, the elementary and composite particles made of the quarks (in purple) and leptons (in green) would be "matter"; while the gauge bosons (in red) would not be "matter". However, interaction energy inherent to composite particles (for example, gluons involved in neutrons and protons) contribute to the mass of ordinary matter.
Tuesday, September 07, 2010
Wednesday, August 25, 2010
Tuesday, August 17, 2010
Saturday, April 17, 2010
Sunday, March 28, 2010
Sunday, January 24, 2010
Moscow’s stray dogs

Neuronov says there are some 500 strays that live in the metro stations, especially during the colder months, but only about 20 have learned how to ride the trains. This happened gradually, first as a way to broaden their territory. Later, it became a way of life. “Why should they go by foot if they can move around by public transport?” he asks.......
Financial Times article: click here
Friday, December 25, 2009
Friday, December 04, 2009
Get those leaks out of your code.
http://msdn.microsoft.com/en-us/library/ee658248.aspx
Satya Musica.
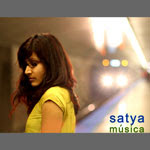